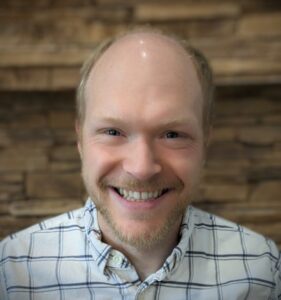
Atomic, molecular and optical and quantum theorist Matthew Otten will join the UW–Madison physics department as an assistant professor on January 3, 2024. He joins us most recently from HRL Laboratories. Prior to HRL, Otten earned his PhD from Cornell University, and then was the Maria Goeppert Mayer fellow at Argonne National Laboratory.
Please give an overview of your research.
Very generally, my goal is to make utility scale quantum computing a reality, and to get there faster than we would otherwise without my help. We have a lot of theoretical reasons to believe that quantum algorithms will be faster in certain areas; in practice, we need to know how expensive it’s going to be. It could be that a back of the envelope calculation says a quantum computer might be better, but because quantum computers are very expensive to build and have a lot of overhead, you could find that once you crunch the numbers really carefully, it turns out to cost more money or more energy or more time than just doing it on a supercomputer. In that case, it’s not worth the investment to build it, or at least not at this point. Part of my research is to understand and develop quantum algorithms and count how expensive they are. Once you do that, you can figure out the reason it’s so expensive is A and B. Then we go and we try to fix A and B, and then whack-a-mole all these bottlenecks down and eventually you go from, “It’ll never work,” to “Okay, it’ll work in twenty years.”
Another part of my research is looking at the physical qubits. These devices all have a lot of deep physics inside of them. If you just look at it from the quantum algorithm level, you might get so far. But if you dig down and try to understand the underlying physics, I think you can get further. You might be able to make devices cheaper, faster, or more performant in general. I do a lot of simulations of the underlying physics of these various types of qubits to understand what their properties are, what causes the noise that ruins computation, and what we can do to fix that noise. Through simulations on classical computers, sometimes very large ones, we come up with ways to tweak the system so that you get better performance, by coming up with better quantum algorithms and better qubits. Put those together and hopefully you get to a better quantum computer.
Once you arrive in Madison, what are one or two research projects you think your group will focus on first?
I’ll be bringing a few projects with me. The first is part of a DARPA program called Quantum Benchmarking, which I was part of while at HRL. We found really high-value computational tasks, not specifically quantum, that Boeing, which owns HRL, would like calculated: for instance, reducing corrosion. Corrosion causes planes to be grounded for maintenance, which is costly. Reducing corrosion will reduce maintenance costs and increase uptime. We’ve been developing ways to ask and answer the question, how close are today’s quantum computers to solving that problem? How big do quantum computers need to be to solve that problem? The specific task is understanding what it takes to solve such a large-scale problem, counting the quantum resources that are necessary and coming up with tests so that you could go to a quantum computer, run the tests, and hopefully be able to predict how much bigger or how much faster they would need to be to solve the problem.
Another one comes from the Wellcome Leap Foundation. We are trying to do the largest, most accurate calculation of biological objects — a molecule, string of carbon, something like this — possible on a real-life quantum computer. We’re trying to take techniques that have already been developed or develop new techniques to make circuits smaller, which means a less expensive quantum computer, and faster. That one is a competition, they gave us funding to do it, but if we complete the task better than other competitors, we get more funding to do more.
What attracted you to UW–Madison?
The strength of the science that’s happening in the physics and broader Wisconsin community is very attractive. When I visited, everyone was very nice, it’s a very collegial department. And being from St. Louis, I like the Midwest. I’ve lived in Southern California for a couple of years now and I haven’t seen snow, and that’s sad. Madison is a lovely area. Great people.
What is your favorite element and/or elementary particle?
I think it has to be silicon. Silicon is used in classical computing and potentially has use in quantum computing. And you’re carrying around silicon right now, just like everyone else.
What hobbies and interests do you have?
I have a Siberian Husky puppy and we’ll be very happy to go to Madison and do a lot of skijoring, which is cross country skiing, but the dog pulls you. I started running recently and I was jazzed up for my first half marathon and then I got COVID and I didn’t do it, so I’m still jazzed up for my first half marathon. I play a lot of board games and have a very large board game collection. And my daughter just turned one. She’s become a new hobby.