If net-positive fusion energy is to ever be achieved, density is key: the more atomic nuclei crashing into each other the more efficient the reaction will be. Nearly 40 years ago, Martin Greenwald identified a density limit above which tokamak plasmas become unstable, and the so-called Greenwald limit has at best been exceeded by a factor of two in the ensuing decades.
In a new study published July 29 in Physical Review Letters, physicists at the University of Wisconsin–Madison produced a tokamak plasma that is stable at 10 times the Greenwald limit. The findings may have implications for tokamak fusion reactors, though the researchers caution that their plasma is not directly comparable to that in a fusion reactor.
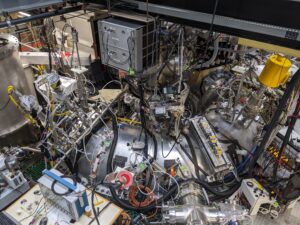
“Tokamak devices are considered a leading contender in the race to build a nuclear fusion reactor that generates power in the same way as the sun,” says Noah Hurst, a scientist with the Wisconsin Plasma Physics Laboratory (WiPPL) and lead author on the study. “Our discovery of this unusual ability to operate far above the Greenwald limit is important for boosting fusion power production and preventing machine damage.”
Tokamaks are toroidal devices, basically hollow metal donuts that churn ionized plasma through the tube by applying both a magnetic field and an electrical current. This shape has been shown to be particularly adept at confining the plasma, which is required to reach the high temperature and density needed for fusion. But the design can also lead to instabilities in the plasma: as its density increases, the plasma becomes more turbulent, causing the plasma to give up all its energy to the wall and cool off.
The device that the WiPPL team used in this new study is the Madison Symmetric Torus, or MST. For many years, MST has operated as one of the leading programs studying the reversed field pinch, a toroidal configuration closely related to the tokamak. MST was designed to anticipate operation as a tokamak, allowing direct comparison of the two toroidal configurations in the same device. Unlike other tokamaks, the metal donut that houses the MST plasmas is thick and highly conducting, allowing for more stable operation..
In 2018, MST scientists received National Science Foundation funding to build power supplies that are programmable, facilitating easier access to a range of toroidal plasma configurations, from tokamak to reversed field pinch. Hurst was hired in 2019 to study MST plasmas in tokamak mode with the new power supply.
“My job was to try to find ways to make the plasma go unstable,” Hurst says. “I tried, and I found that, well, in many cases, it doesn’t. It was surprising.”
The Greenwald limit is just the ratio of the plasma density to the product of the plasma current and plasma size, a simple metric that allows comparison of different devices and operating conditions. Since the limit was defined, only a handful of devices have operated above it, and by at most a factor of two.
“Here, we were at a factor of ten,” Hurst says. “Future reactor-scale tokamaks will likely need to operate near or above the Greenwald limit, so if we can better understand what’s causing the density limit and understand the physics of how we got to ten times the limit, then maybe we have a shot at doing something about it.”
Though the researchers feel confident in their results, they are unexpected. The team is actively exploring explanations.
“The first thing we would ask is, what’s different about our machine relative to other machines?” Hurst says. “MST is very different because it was designed with a thicker wall than most tokamaks. Also, most tokamaks produce lower-resistance plasmas, so they don’t need these large voltages like we did in order to run.”
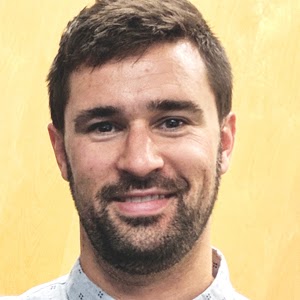
Hurst also emphasizes that these results are unlikely to be directly applicable to fusion reactors, such as ITER and others that are being built in the hopes of being the first net-positive energy production tokamaks. But he and the team are cautiously optimistic.
“Our results were obtained in a low magnetic field, low temperature plasma, which is not capable of fusion power production. Still, we were the first ones to be able to do this, and you have to start somewhere,” Hurst says. “We’re going to keep studying these plasmas, and we think that what we learn might help higher-performance fusion devices to operate at the higher densities they need to be successful.”
This study was supported by the U.S. Department of Energy (DE-SC0020245); by the Wisconsin Plasma Physics Laboratory, a research facility supported by the U.S. DOE Office of Fusion Energy Sciences under contract DE-SC0018266; and by a National Science Foundation Major Research Instrumentation grant (PHY 1828159).