This post is adapted from an Astronomical Society of the Pacific press release
The Astronomical Society of the Pacific (ASP) has awarded the 2022 Catherine Wolfe Bruce Gold Medal to Ellen Zweibel. It is the most prestigious award given by ASP.
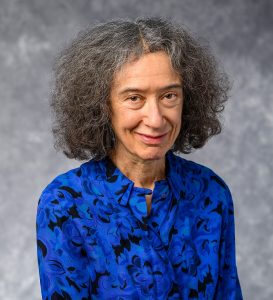
Zweibel, the William L. Kraushaar professor of astronomy and physics at UW–Madison, was recognized for her contributions to the understanding of astrophysical plasmas, especially those associated with the Sun, stars, galaxies, and galaxy clusters. She has also made major contributions in linking plasma characteristics and behaviors observed in laboratories to astrophysical plasma phenomena occurring in the universe.
Most plasma effects in astrophysical systems are due to an embedded magnetic field. Many of them can be grouped into a small number of basic physical processes: how magnetic fields are generated, how they exchange energy with their environments (sometimes on explosively fast timescales), their role in global instabilities, how they cause a tiny fraction of thermal particles to be accelerated to relativistic energies, and how they mediate the interaction of these relativistic particles (cosmic rays) with their gaseous environments through waves and instabilities on microscales. Although all these processes occur in laboratory plasmas, it is in natural plasmas that they take their most extreme forms. Zweibel and her students and postdocs have used analytical theory and numerical simulations to study the generation and evolution of magnetic fields in the Sun and other stars, in galaxies, and in galaxy clusters, and have researched the effects of high energy cosmic ray particles in all of these environments. Their most recent work centers on the role of cosmic rays in star formation feedback: the self-regulation of the star formation rate in galaxies through energy and momentum input to the ambient medium by the stars themselves.
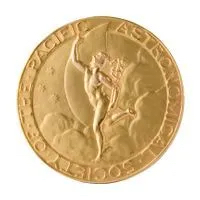
Zweibel has authored over 242 refereed publications with over 8,000 citations. In 2016 she was awarded the American Physical Society’s James Clerk Maxwell Prize for Plasma Physics “For seminal research on the energetics, stability, and dynamics of astrophysical plasmas, including those related to stars and galaxies, and for leadership in linking plasma and other astrophysical phenomena.” She is a member of the National Academy of Sciences.
The Astronomical Society of the Pacific’s Catherine Wolfe Bruce Gold Medal was established in 1898 by Catherine Wolfe Bruce, an American philanthropist and patroness of astronomy. The ASP presents the medal annually to a professional astronomer in recognition of a lifetime of outstanding achievement and contributions to astrophysics research. It was first awarded in 1898 to Simon Newcomb. Previous recipients of the Bruce Medal include Giovanni V. Schiaparelli (1902), Edwin Hubble (1938), Fred Hoyle (1970), and Vera Rubin (2003)