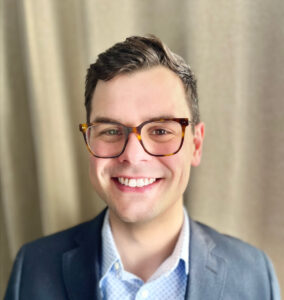
Condensed matter theorist Ben Woods joined the department as an assistant professor this fall. Originally from a small town in North Dakota, Woods studied physics at the University of North Dakota and earned a PhD in physics from West Virginia University. He first came to UW–Madison for a postdoc with Mark Friesen in 2021, and now moves into his faculty role.
Please give an overview of your research.
I primarily work in two main areas of condensed matter theory and quantum information science. The first area is the theory of semiconductor quantum dots, with applications towards building and operating quantum computers based on spin qubits. Quantum dots can be thought of as artificial atoms in which electrons are trapped and manipulated within a semiconductor, such as silicon, by metallic gates that sit on top of the semiconductor. An electron in the quantum dot forms the basis for a type of qubit called a spin qubit, where the quantum information is stored in the spin of the electron. I investigate how we can build higher quality spin qubits. One aspect of this is analyzing and designing single and two qubit gates such that their efficiency and noise resiliency can be improved. Another aspect is studying the materials and design of quantum dot devices to optimize certain properties, such as how the qubits respond to an external magnetic field. I am also interested in quantum dot arrays as a platform for quantum simulation. Here the idea is to engineer the interactions between the quantum dots to emulate a quantum system of interest.
The other area I work in is semiconductor-superconductor heterostructures. Here, you’re trying to combine desirable properties of both types of materials to create interesting devices that would otherwise be impossible. I study semiconductor-superconductor heterostructures that can give rise to exotic particles known as Majorana zero modes, which form the basis for topological qubits. These qubits are immune to certain error sources that more conventional types of qubits are not. I am trying to understand the effects of disorder on these heterostructures and develop new schemes in which Majorana zero modes can be realized.
What are one or two of the main projects your group will work on first?
One initial project will focus on designing a new qubit architecture for quantum dot spin qubits. In the most conventional type of spin qubit, you have a single electron spin that is manipulated by jiggling it with an electric field back and forth within a single quantum dot. It turns out, however, that these qubits can be manipulated more efficiently if you can hop electrons between multiple quantum dots. Specifically, I’ve devised new schemes involving three dots in a triangular geometry in which single-qubit gates can be performed quite efficiently. These ideas work in principle, but now it’s a matter of quantitatively studying how noise resilient the scheme is and how finely tuned the system parameters need to be for things to go as planned.
A second initial project is more towards quantum simulation using quantum dot arrays. The project will focus on studying magnetism in quantum dot arrays. In other words, asking how the spins of the quantum dot electrons organize due to their mutual interaction. One interesting wrinkle in these quantum dot arrays based on silicon is that there is a valley degree of freedom in addition to the usual spin degree of freedom. The project involves understanding the effects on the magnetic ordering due to this additional valley degree of freedom. Specifically, I am interested in how fluctuations in the valley degree of freedom from one dot to the next can impact magnetic ordering.
What attracted you to Madison and the university?
There were two main reasons. First, my wife had gotten a residency as an anesthesiologist at the UW hospital. So that was an obvious motivation. Second, one of my grad school advisors knew Mark Eriksson and Mark Friesen and thought it’d be a natural fit for me to work with them as a postdoc. Since moving here, my family has enjoyed Madison, and I really like the physics department. The people are very friendly and collaborative. I am incredibly happy to be able to stay in Madison and at the UW physics department.
What is your favorite element and/ or elementary particle?
It has to be silicon, right? It’s the material I think about every day. And the world economy is largely based on stuff made with silicon. So that’s pretty cool?
What hobbies and interests do you have?
I like to play guitar, read, watch sports, and spend time with my family and friends. I have two kids, three years and six months old, who I like to spend most of my free time on.